⇐ Previous Article — Table of Contents — Next Article ⇒
New Energy Times home page
12. Weak Interactions Are Not Weak Energetically
By Steven B. Krivit
From the perspective of physics, four fundamental forces exist in our world: gravity, electromagnetism, strong interactions and weak interactions.
From birth, we learned about gravity; it became obvious the moment we tried to walk for the first time. Eventually, we were old enough to open the refrigerator door. It required a bit of a tug. And when we closed it, there was a bit of a snap when the door came within a few millimeters of the refrigerator frame. For those of us who liked to take things apart, we found that magnets lined both the door and the frame. Thus, we learned about electromagnetism.
People who went on to become nuclear engineers and physicists learned about strong-force interactions; these are the fundamental forces which enable nuclear fusion to occur or, conversely, they inhibit nuclear fission from occurring.
Weak interactions, though, are relatively new to science. Around 1968, physicists Sheldon Glashow, Abdus Salam and Steven Weinberg developed a broad theory of weak interactions at high energies – that is, weak-energy theory applied to high-energy nuclear research experiments. They won a Nobel Prize for their work in 1979.
But experimental verification of weak interactions didn't occur until the 1980s. Much of the related work took place at CERN, the European Organization for Nuclear Research. Carlo Rubbia, for example, former president of ENEA, the Italian National Agency for New Technologies, Energy and the Environment, won a Nobel Prize for related weak-interaction work in 1984.
When "cold fusion" came along in 1989, people were confused. It clearly wasn't strong-interaction fission. And it certainly seemed like a strange variant of thermonuclear fusion, also a predominately strong-interaction process.
Very few people who looked at "cold fusion" early on – proponents and skeptics alike – knew about weak interactions. Those people who did have some familiarity with weak interactions didn't consider that these processes might be fundamentally responsible for LENR phenomena. Before Allan Widom and Lewis Larsen released the pre-print of their LENR theory in 2005, most people thought that weak interactions had no capacity for significant energy release.
Larsen describes weak interactions simply as "any type of nuclear process that either emits or absorbs a neutrino," for example, beta decays or beta-delayed alpha decays. Weak interactions are not necessarily weak energetically, as he wrote in 2008 for the U.K. Institute of Science in Society[1]:
In the aftermath of World War II, Enrico Fermi’s beloved weak interactions became somewhat neglected. It was looked upon more as a scientific curiosity of theoretical interest with no practical applications. After all, every physicist and chemist “knows” that a) radioactive beta decay rates are mainly low-energy and, b) being random, they cannot be controlled; and hence c) they are useless for power generation applications.
Also, no one considered the possibility of creating neutrons directly via the weak interaction; there just didn’t seem to be any reasonable way to get weak interaction rates high enough to be useful. The Widom-Larsen theory of LENRs and hundreds of credible experiments have demonstrated otherwise.
Widom and Larsen applied the existing electro-weak theory of the Standard Model and added many-body collective effects.
Larsen explains that, "contrary to common belief, weak-interaction LENRs are not necessarily weak in terms of the total amount of energy released."
Widom and Larsen provide an example of a series of ultra-low-momentum neutron-catalyzed LENR processes that start with lithium, in which weak interactions are very important[2]:
Lithium-6 + 2 neutrons ⇒ 2 helium-4 + beta particle + neutrino + 26.9 MeV
This particular series can release about the same amount of energy as fusion reactions without creating any energetic neutrons, hard gamma radiation, or hot radioactive isotopes. While some of the 26.9 MeV in excess nuclear binding energy released is certainly lost to the neutrino, much of it remains in the kinetic energy of the two helium atoms (alpha particles) and beta particle.
Local solid matter is heated-up by the impacts of the alpha and beta particles; and heavy-mass electrons also convert any locally produced hard gamma or X-rays directly into infrared heat.
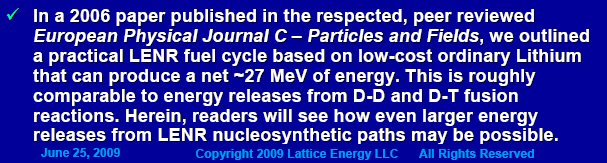
Source: June 25, 2009 Slide Presentation by Lewis Larsen
In another article he wrote for the Institute of Science in Society[3], Larsen explains the potential energy yield from LENR. According to the Widom-Larsen theory, fuels used in LENR have the capacity to release several thousand times more energy than gasoline does. The following text is a slightly edited version of what Larsen wrote in the article:
The calculation of energy yield for a prospective LENR target fuel is straightforward. It involves two steps. The first is to decide which “base fuel” (hydrogen or deuterium) to use for producing LENR neutrons that can then react with a prospective target fuel. This choice determines the energy “cost” of the necessary neutrons. The second step is to calculate the nuclear [binding] energy released by a particular target fuel when it subsequently absorbs (captures) the neutron(s).
It “costs” a minimum of 0.78 MeV of input energy to make a heavy-mass electron (e*) that has enough additional effective mass-energy to successfully react with a given “base fuel” such as hydrogen H = (p) or deuterium D = (p,n) to produce the ultra-low-momentum (ULM) neutrons (n). The required input energy that is “pumped” into the collective plasmon polariton electrons found on all metallic hydride surfaces can be in the form of an externally imposed electric current, laser pulse, injection of energy into a local magnetic field, pressure gradient across a metallic hydride “membrane,” and so on.
The minimum input energy “cost” to make a heavy electron (e*) is exactly the same, 0.78 MeV, regardless if H or D is used. While D is a much more expensive “base fuel” than is ordinary hydrogen, it is still an attractive option for many commercial LENR applications because it produces two LENR ultra-low-momentum (ULM) neutrons for the input energy “cost” of one[4], so the cost per ULM neutron is just 0.39 MeV for D instead of 0.78 MeV for H.
If, upon absorption of neutrons by a given target fuel atom, subsequent reactions release somewhat more (losses to neutrinos must be factored in) than 0.78 MeV (using H) or 0.39 MeV (using D) per neutron absorbed, then the LENR fuel burn-up process exceeds breakeven. That is, you are then ahead of the game, at least as far as the overall energetics is concerned.
The energy range of individual beta decays extends from keVs up to about 20 MeV for certain neutron-rich isotopes[5]; many potential LENR target fuel beta decay processes are well-above the breakeven point. The multi-step LENR reaction series is outlined in our 2006 paper.[1] |
For a high-level overview of the full Widom-Larsen theory, please see "Widom-Larsen Theory Simplified" in this special report.
Widom and Larsen are not the only LENR theorists familiar with the potential power of weak interactions.
Xing Zhong Li, professor emeritus from Tsinghua University in China, with a long career in nuclear physics behind him, stated as much in a paper about his own theory, a resonant tunneling model.[6]
"The weak interaction does not mean a weak power source," Li wrote. "Even if the lifetime is as long as 104 seconds (~3 hours), a cubic centimeter of palladium might produce megawatts of thermal power. Its power density is higher than that of the fuel rod in a fast fission reactor."
References
1. Larsen, L., "Widom-Larsen Theory Explains Low-Energy Nuclear Reactions and Why They Are Safe and Green," Institute of Science in Society Report, April 12, 2008.
2. Widom, A., and Larsen, L., "Ultra-Low-Momentum Neutron-Catalyzed Nuclear Reactions on Metallic Hydride Surfaces," European Physical Journal C - Particles and Fields, Vol. 46(1), p.107 (2006).
3. Larsen, L., "Portable and Distributed Power Generation from LENRs," Institute of Science in Society Report, Oct. 12, 2008.
4. Larsen, L., Slide presentation, June 25, 2009.
5. Larsen, L., Slide presentation, Sept. 3, 2009.
6. Li, X.Z., Wei, Q.M., and Liu, B., "An Approach to Nuclear Energy Without Strong Nuclear Radiation," Department of Physics, Tsinghua University, Beijing 100084, China, in Low-Energy Nuclear Reactions Sourcebook, Marwan, J., and Krivit, S,B., eds., American Chemical Society/Oxford University Press: Washington, D.C., ISBN 978-0-8412-6966-8, August 2008.
⇐ Previous Article — Table of Contents — Next Article ⇒
|